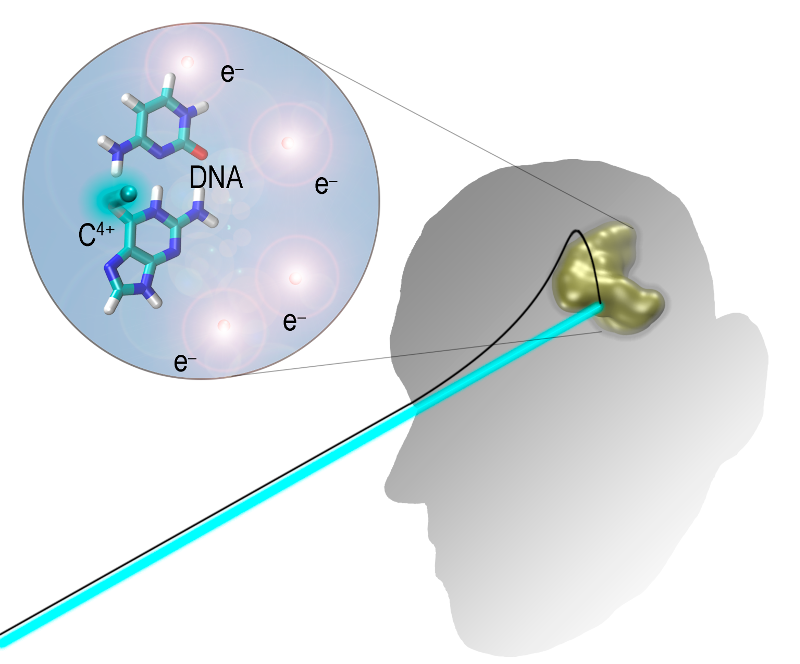
A century ago, radiation therapy emerged as a significant tool in oncology. Proton beam experiments for cancer treatment commenced in the late 1950s. Still, it wasn't until 1990 that this approach was integrated into clinical practice. Over time, researchers recognized the potential of heavier particles, such as carbon nuclei, in delivering more effective treatment. This realization led to developing Ion Beam Cancer Therapy (IBCT), a sophisticated modality demonstrating increasing efficacy in treating specific cancer types.
At a microscopic scale, envision minuscule carbon particles within the beam engaging with molecules within cancer cells. These interactions precipitate the generation of secondary electrons, which serve as agents responsible for inducing damage to the cancerous cells. Remarkably, this intricate process unfolds within a few hundred attoseconds, highlighting its swift nature. Notably, collisions between carbon ions and water molecules play a pivotal role in this intricate dance of interactions.
Our focus is exploring the collisions between carbon ions and DNA in cancer cells. This pursuit offers an opportunity to delve into the quantum mechanical dynamics governing these events with precision. We aim to unravel the complexities underlying Ion Beam Cancer Therapy at a fundamental level. By wearing these intricacies, we strive to enhance our comprehension of this cutting-edge therapy, potentially paving the way for advancements in cancer treatment methodologies.